Divorce in a one-dimensional world
Scientists at the Max Planck Institute of Quantum Optics have experimentally confirmed a fifty year old prediction about the (in)separability of an electron's spin and electric charge using a specialised quantum simulator. The result is now published in "Science".
In objects of our everyday world, there is no left without right or front without back. Just as inseparable seem to be the electron's electric charge and its "spin". But in a strictly one-dimensional quantum world, both quantum properties are separable from each other. This fifty-year-old prediction has now been confirmed by an experiment conducted by a team from the Munich Center for Quantum Science and Technology (MCQST). Physicists from the Max Planck Institute for Quantum Optics in Garching are playing a leading role. For their successful demonstration, which is now published in the journal “Science”, they used a so-called quantum simulator. Such a specialized quantum computer can precisely estimate the quantum properties of a material, which is impossibly challenging for conventional supercomputers today.
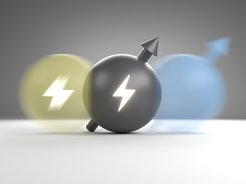
"As a scientist, when you think of an electron, you think of a bound unit with a certain electrical charge and a certain spin," explains Jayadev Vijayan, PhD student in the group of Christian Gross and Immanuel Bloch, director at the Max Planck Institute of Quantum Optics in Garching. Spin can be imagined as a kind of quantum mechanical gyroscope. But it is much more, because its special spin turns an electron into a "Fermion" with quantum properties that provide also the foundation of today’s semiconductor electronics.
Therefore, the charge and spin of an electron are considered to be inseparably connected. But more than fifty years ago scientists came to a surprising realization that in a strictly one-dimensional world, this trusted togetherness of charge and spin could be separable. If you pull an open pearl necklace straight as an arrow, you've basically created such a one-dimensional world. In the experiment, the pearls are magnetic atoms. In each of these atoms there is a special electron whose unshielded spin turns the atom into a small magnetic needle. Since the opposite poles of the magnetic needles attract each other, neighbouring magnetic needle beads on the string align in opposite directions: a north pole points in one position upwards, the next neighbouring north pole downwards, then next but one upwards again, so it is a chain of alternating spins pointing up and down (see figure).
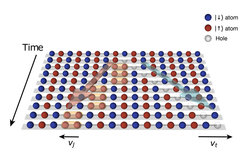
This is starting point of the experiment. The prediction now says: If such a one-dimensional quantum pearl chain is disturbed, then the charge and the spin of an electron can separate from each other in an atomic pearl. Then both should run along the chain as two separate "quasiparticles". These quasiparticles can be imagined as a bucket of water and a bucket of sand, which are passed on at different speeds in a fire extinguishing chain.
The Munich team also faced an experimental challenge. Today's nanotechnology can produce one-dimensional atomic "pearl chains". "But the electrons are separated by a distance in the order of a tenth of a nanometer," explains the PhD student. Roughly one tenth of a billionth of a meter is typical for the distance between atoms in materials. This is too tiny to observe under a microscope, making it impossible to study their behaviour.
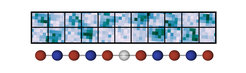
Graphic B shows the state of the chain after the quench (lower row) in the microscope image. Atoms with an up spin end up in the upper row, the atoms with down spin end up on the lower row and sites with no signal contain holes.
The quantum simulator
This is where the Munich quantum simulator comes into play. In principle, it works like replacing the string of the pearl necklace with a rubber band. And the rubber band is pulled apart so that the distance between the atomic beads is roughly ten thousand times greater. This micrometer range can now be resolved by a light microscope. The tiny atoms become visible when laser light causes them to light up. In the experiment, the "rubber band" consists of a grid of intersecting laser light beams. Each light intersection acts like a small trap that captures an atom, in this case a lithium atom. To make them behave like electrons in real materials, they must first be cooled to ultra-low temperatures in vacuum.
Lithium atoms are fermionic, i.e. small magnets carried by an unshielded electron spin. Now the physicists had to come up with a trick to make this spin visible in their quantum simulator. To do this, they "loosen" the shackles of light for a short time in the presence of a specially designed magnetic field. The result: The atoms shear slightly upwards or downwards from the pearl chain, depending on the direction of their spin.
Feynmans Dream
As soon as the chain of atoms is prepared, the physicists kick an atom out of the middle of the chain with laser light. This disturbance, called "quench", creates two quasiparticles in the chain. The first quasiparticle is the hole left by the ejected atom. This "holon" contains the quantum property of the electron charge. The second quasiparticle, called the spinon, consists of the two adjacent parallel spins left behind by the holon gap. Compared with the background of alternating spins pointing up and down, this spinon carries an excess spin from the quench.
With their quantum simulator, the team was able to follow exactly how the two perturbations travel along the atomic chain. In fact, it turned out that they move at different speeds and not bound together. Charge and spin are thus completely independent of each other and perfectly separated - just like the water and sand buckets in the fire extinguishing chain.
On the one hand, this result is exciting from the perspective of basic research in quantum physics. The separability of charge and spin could one day also find fascinating applications in quantum information technology. Above all, however, the Garching experiment successfully demonstrates that quantum simulators are developing into a technology to be taken seriously. Already in the 1980s, the famous Nobel Prize winner Richard Feynman dreamed that it would be possible to understand the behaviour of the materials' quantum systems, which are hard to access experimentally, by using analogous quantum systems that were perfectly accessible and controllable. Even conventional supercomputers fail to calculate some of such quantum systems exactly. But this elegant possibility is offered by ultracold atoms in light grids. "In the future, this could enable the targeted design of new materials that, for example, become superconducting at room temperature," says Jayadev Vijayan. Feynman's dream of a quantum simulator is now becoming reality.
(RW)