Research Details
Contents:
Molecular ensembles at sub-Kelvin temperatures
- Velocity filtering: simplicity often is best
- Buffer-gas cooling: precooling molecules with cryogenic helium
- Centrifuge deceleration: enhancing gravity isn’t science fiction
- The Cryofuge: combining buffer-gas cooling with centrifuge deceleration
- A microstructured electric trap: holding onto cold molecules for over a minute
Optoelectrical Sisyphus cooling: bridging the gap to ultracold temperatures
- Cooling molecules by replacing photon recoil with electric field interactions
- Initial demonstration of optoelectrical cooling on methyl fluoride
- Formaldehyde molecules at submillikelvin temperatures
Internal state control of polar molecules
- Measuring internal state distributions via depletion techniques
- Cooling internal degrees of freedom via optical pumping on a vibrational transition
Interfacing polar molecules with Rydberg atoms
Molecular ensembles at sub-Kelvin temperatures
Most quantum experiments with polar molecules require trapped molecules since trapping dramatically increases the level of control compared to free space beams. As the trap depth which can be achieved when trapping molecules using electric fields is roughly 1K×kB, a key step for all our experiments is the production of an initial molecule ensemble with an energy below this value. For this purpose, our group has pioneered two successful approaches, velocity filtering and buffer-gas cooling in combination with centrifuge deceleration.
Velocity filtering: simplicity often is best
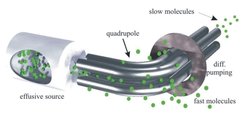
A particularly simple approach to obtaining cold molecules is based on the consideration that even at room temperature, the thermal distribution of molecule velocities includes a certain fraction of molecules which is slow at any instance in time. Cold molecules can be obtained by separating these slow molecules from the fast ones. This idea of filtering slow molecules out of a thermal gas in fact goes back to experiments of Zacharias in the 1930's, but was unsuccessful at the time due to technical challenges.
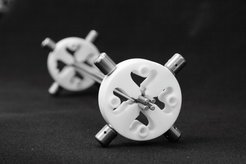
In our experiments, four high-voltage electrodes forming an electric quadrupole potential allow slow molecules in low-field-seeking states to be trapped in two dimensions. Transversely slow molecules from an effusive beam source of molecules can thereby be separated from fast ones, with fast molecules eliminated by a high-speed pump. Longitudinally slow molecules are separated from longitudinally fast ones via a bend in the guide.
After initially demonstrating this technique in 20031, we have performed a wide range of experiments to understand and perfect velocity filtering. This includes using ac rather than dc fields for guiding allowing a larger variety of molecular states to be guided2, understanding collisional effects which limit the density of cold molecules which can be obtained3, investigating different water isotopologs which beautifully illustrates the effect of the internal molecule structure on guiding properties4, and generation of molecule pulses with particularly narrow velocity distributions by appropriately switching consecutive segments of the quadrupole guide5. At this point, velocity filtering serves as a well understood workhorse for further experiments.
1S.A. Rangwala et al., Physical Review A 67, 043406 (2003)
2T. Junglen et al., Physical Review Letters 92, 223001 (2009)
3M. Motsch et al., Physical Review A 79, 013405 (2009)
4M. Motsch et al., New Journal of Physics 11, 055030 (2009)
5C. Sommer et al., Physical Review A 82, 013410 (2010)
Buffer-gas cooling: precooling molecules with cryogenic helium
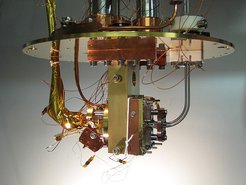
While velocity filtering works great for smaller molecule species with a limited number of internal states populated in the source, using this method to obtaining full quantum control over larger molecules, with millions or more internal states populated at room temperature, is exceedingly challenging. To enable us to work with a wider range of molecule species, we replace the effusive beam source in velocity filtering with a cryogenic buffer-gas source. Here, molecules thermalize to the cryogenic environment via collisions with helium or neon buffer-gas. This not only cools the molecule’s motional degrees of freedom, allowing a larger fraction of molecules to be captured by the quadrupole electric guide, but also cools the internal degrees of freedom, resulting in an ensemble with a much higher internal-state purity.
Due to the complicated hydrodynamics at cryogenic temperatures taking place inside the buffer-gas cell, finding optimal operating parameters has been a considerable challenge. For this purpose we have used a number of tools, including a random-walk collision model to simulate the cell dynamics at low buffer-gas density, a full hydrodynamics model to simulate the cell dynamics at high buffer-gas density, comprehensive simulations of the electric guiding of molecules outside the buffer-gas cell, and RF and UV depletion methods to measure molecule’s internal state populations.
L.D. van Buuren et al., Physical Review Letters 102, 033001 (2009)
C. Sommer et al., Faraday Discuss. 142, 203 (2009)
X. Wu et al., ChemPhysChem 17, 3631 (2016)
Centrifuge deceleration: enhancing gravity isn’t science fiction
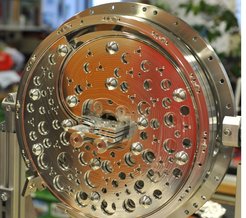
A fundamental problem of buffer-gas cooling of molecules confronting all groups using this method worldwide is that collisions of the molecules with the buffer-gas at the buffer-gas cell exit deplete the slowest molecules from the resulting molecular beam. This eliminates essentially all molecules with a translational energy below the roughly 1K which we require for further applications. Transforming buffer-gas cooling into a practical source of cold molecules thus requires deceleration of the resulting molecular beam.
One approach to decelerate a molecular beam would be to launch the molecules upward in a vertical shaft or tower, allowing them to be decelerated by the earths gravitational field. This would require a vertical distance of about 125m and 4500m for an initial molecule velocity of 50m/s and 300m/s, respectively. To keep the experiment laboratory-sized, we effectively enhance gravity using the centrifugal potential in a rotating reference frame. Molecules are injected at the periphery onto a disk rotating at up to 100Hz. A quadrupole electric guide mounted on the rotating disk confines the molecules to the rotating frame and guides the molecules to the center of the disk where they are extracted along the axis. In the process, the molecules are forced to climb the centrifugal potential, allowing us to decelerate molecules moving at up to about 200m/s over a radial distance of 20cm.
S. Chervenkov et al., Physical Review Letters 112, 013001 (2014)
The Cryofuge: combining buffer-gas cooling with centrifuge deceleration
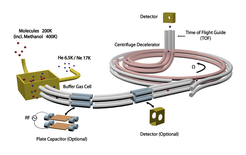
Whereas initial experiments with the centrifuge were performed using velocity filtering as an initial source of molecules, realizing the full potential of the centrifuge requires combining it with the cryogenic buffer-gas cell. We have thereby realized an ideal source of cold molecules which are simultaneously internally cold, slow, and dense. We obtain fluxes of molecules with a translational energy below 1K×kB with a high internal state purity exceeding 1010/s, which is orders of magnitude higher than for previous sources of cold molecules. Moreover our molecule source is applicable to a broad variety of cold molecule species. To date we have achieved slow beams for a total of 5 different molecule species: deuterated ammonia (ND3), methyl fluoride (CH3F), trifluoromethyl acetylene (CF3CCH), isopropanol (C3H8O), and methanol (CH3OH), and a wide range of additional species would be equally possible. The cryofuge thus provides perfect conditions for further experiments, and in fact directly allowed us to perform first collision experiments in a quadrupole electric guide.
X. Wu et al., Science 358, 645 (2017)
A microstructured electric trap: holding onto cold molecules for over a minute
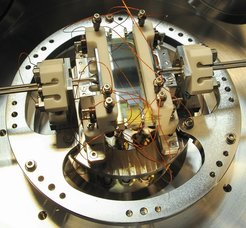
Once a molecule ensemble’s translational energy is reduced below roughly 1K in all three dimensions, the key next step is to load the molecules into an electrostatic trap, greatly extending the timescale on which the molecules can be used for further experiments. To optimize the attributes of the trap to the greatest extent possible, we have devised a very unique trap design based on trapping molecules between a pair of microstructured capacitor plates with an additional perimeter electrode for transverse confinement. The microstructure creates strong electric fields near the plate surface which however decay exponentially away from the plate surface. This results in strong confinement for the molecules while simultaneously allowing the capacitor plates to create a tunable homogeneous electric field in the center of the trap volume. We thereby obtain a roughly box-shaped potential for the molecules.
Based on our unique design, we achieve three important goals. First, the geometry of our trap allows connection to and continuous loading of molecules from a quadrupole electric guide. This allows our trap to be seamlessly connected to the sources of cold molecules described above and allows us to accumulate large ensembles of molecules from these sources over many seconds. Second, the homogeneous electric fields in the trap center allow molecule transitions to be driven with electromagnetic fields with a linewidth which is orders of magnitude lower than it would be otherwise due to Stark broadening. This is essential for the further experiments described below. Finally, our trap provides record lifetimes for polar molecules. A trap lifetime of over 10s with storage for up to a minute was achieved during initial experiments. This lifetime was subsequently extended to almost a minute (56.7±2.4s), which is the longest trap lifetime for storing cold molecules in any experiment worldwide. Our trap thus provides ideal conditions for a wide range of further experiments, as described below.
B.G.U. Englert et al., Physical Review Letters 107, 263003 (2011)
Optoelectrical Sisyphus cooling: bridging the gap to ultracold temperatures
All of the techniques presented thus far focus on producing and trapping cold molecules at a temperature of roughly 1K. Most of the applications mentioned in the introduction require substantially lower temperatures, however, and thus require a second stage cooling method. For this purpose our group has pioneered a new approach: optoelectrical Sisyphus cooling.
Cooling molecules by replacing photon recoil with electric field interactions
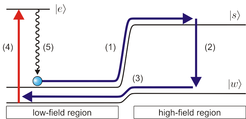
The production of cold and ultracold gases was initially investigated for atomic rather than molecular systems, where the standard approach for achieving low temperatures is laser cooling. Laser cooling works by scattering a large number of photons from a laser beam propagating opposite to a particle’s velocity, whereby the small momentum kicks from the scattered photons reduce the particle’s energy. Applying this method to most molecule species is difficult to impossible since the required laser frequency depends on the particle’s internal state, and a molecule can quickly get lost in the huge number of rotational and vibrational states.
To cool molecules efficiently, we replace the photon recoil in laser cooling with electric field interactions to reduce the molecules’ energy, greatly reducing the number of photons which need to be scattered. Molecules are initially in rotational states such that the molecules’ electric dipole moment is strongly aligned antiparallel with respect to an externally applied electric field. When such molecules move from a region with weak electric field to a region with strong electric field due to their thermal motion, they lose kinetic energy due to the electric field interaction. Near the top of the electric field “hill”, a radiofrequency field changes the molecules’ state such that the dipole moment is weakly aligned antiparallel to the electric field. This reduces the strength of the electric field interaction and reduces the height of the electric field “hill”: when the molecules role back down the hill, they regain less energy than they originally lost. Once the molecules are back in the weak electric field region, an infrared laser transfers them back to the strongly aligned state and the cycle can be repeated until the molecules’ kinetic energy has been sufficiently reduced. The name Sisyphus cooling for this type of cooling scheme comes from the Greek hero Sisyphus, who was condemned for eternity to role a large stone up a hill only to have it role back down.
M. Zeppenfeld et al., Physical Review A 80, 041401(R) (2009)
Initial demonstration of optoelectrical cooling on methyl fluoride
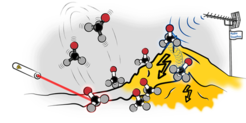
We achieved first experimental results on optoelectrical Sisyphus cooling in 2012 by cooling the symmetric top molecule methyl fluoride (CH3F) with this method. Cold CH3F molecules were produced via velocity filtering and loaded into our electric trap. Applying appropriate infrared, microwave, and radiofrequency fields allowed us to implement the Sisyphus scheme. In this way we were able to reduce the temperature by an additional factor 14 to 29mK. Our result was particularly noteworthy because it was the first time anyone achieved additional cooling of molecules in all three dimensions beyond what is possible in the initial source.
M. Zeppenfeld et al., Nature 491, 570 (2012)
Formaldehyde molecules at submillikelvin temperatures
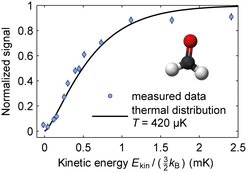
While cooling of molecules to sub-Kelvin temperatures was achieved in 1998, cooling molecules to temperatures below 1mK for many years seemed to be an insurmountable threshold. We finally crossed this threshold in parallel with the group of Dave DeMille at Yale (who use a different method) in 2016 by applying optoelectrical Sisyphus cooling to formaldehyde (H2CO). In comparison to methyl fluoride, formaldehyde has more favorable properties for cooling, in particular an excited vibrational state with a shorter lifetime which allows for faster cooling. Together with a long list of technical improvements, this allowed us to dramatically reduce losses during cooling, and thus cool to much lower temperatures.
As was the case for methyl fluoride, we load velocity filtered formaldehyde molecules into our electric trap. In contrast to methyl fluoride, cooling proceeds at a considerably higher rate, and we substantially extended the cooling sequence. Molecules are thereby cooled by a factor of 1000, allowing us to obtain roughly 300,000 molecules at a temperature of about 400μK. We thus obtain the coldest formaldehyde molecules on earth. Moreover, our molecule ensemble remains the largest ensemble of ultracold molecules obtained in any experiment worldwide.
A. Prehn et al., Physical Review Letters 116, 063005 (2016)
Internal state control of polar molecules
In comparison to atoms, molecules possess a much richer internal energy level structure. Thus, whereas internal structure of atoms is limited to the electronic and nuclear spin degrees of freedom (where the motion and spin of the electrons can be considered separately), in molecules the motion of the nuclei relative to one another leads to additional structure in the form of vibrational and rotational states. Controlling all these internal degrees of freedom is a key prerequisite for quantum applications of polar molecules.
In our experiments, the molecules typically start out at room temperature. Due to their large excitation energies, vibrational and electronic states are then frozen out: only the electronic and vibrational ground state is thermally populated. However, at room temperature, thousands or even millions of rotational and hyperfine states are populated, and we have invested substantial effort to get these states under control.
Measuring internal state distributions via depletion techniques
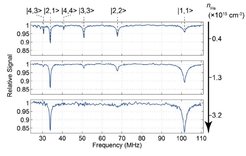
An essential first step for controlling the internal molecular states is the ability to determine the state of our molecules. This is nontrivial in our experiments because we use a general-purpose quadrupole mass spectrometer for molecule detection, which is insensitive to the molecular state. For trapped or guided molecules, state information can nonetheless be obtained via state selective depletion. Molecules in a specific rotational state are state-selectively transferred to an untrapped (or non-guided) state, causing those molecules to be lost from the trapped (or guided) molecule ensemble. The difference in signal with and without the depletion being applied corresponds to the number of molecules in the state of interest.
Over the years, we have used depletion to determine molecule state populations in a number of different settings. For formaldehyde (H2CO), we were able to apply depletion directly in a quadrupole electric guide by driving electronic transitions with a UV laser at about 330nm1. However, this method is difficult to apply to other molecule species with less favorable electronic states. Our microstructured electric trap greatly expanded the possibilities for depletion, allowing us to perform depletion with microwave and infrared radiation to drive rotational and vibrational transitions, respectively2. The homogeneous fields inside the trap allowed us to determine not just the rotational state but also the rotational M-sublevel2. Finally, in order to determine the rotational state of molecules from the cryosource already before installing an electric trap, we replaced a short segment of the quadrupole electric guide with a pair of capacitor plates. This allowed us to perform depletion by driving radiofrequency transitions between rotational M-sublevels3.
1M. Motsch et al., Physical Review A 76, 061402 (2007)
2R. Glöckner et al., New Journal of Physics 17, 055022 (2015)
3X. Wu et al., ChemPhysChem 17, 3631 (2016)
Cooling internal degrees of freedom via optical pumping on a vibrational transition
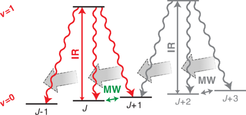
Optical pumping is a well-known tool in atomic physics for controlling atomic states, developed in the 1950s and recognized with the Nobel prize in physics in 1966. For an excited atomic state which decays radiatively to a number of different long-lived lower lying states, driving transitions with radiation to the excited state from all but one of the lower lying states causes an atom which is initially in any of the lower states to end up in the specific state (the so-called dark state) which is not coupled to the excited state. This allows the atom to be prepared in a well defined internal state, i.e., the internal state of the atom is cooled.
Applying optical pumping to molecules is much more difficult than for atoms. For electronic excitations, molecules decay to a much larger number of states than is the case for atoms due to the additional vibrational and rotational degrees of freedom. Even more extreme, for many of the molecules we use, electronic excitation causes the molecule to fracture rather than to decay radiatively.
An alternative option is to excite molecules vibrationally rather than electronically. While vibrational states decay much more slowly than electronic states (typically on many millisecond rather than sub-microsecond timescales), the long trap lifetimes in our microstructured electric trap allows us to wait sufficiently long for decay to occur. Using optical pumping on a vibrational transition we were able to demonstrate internal-state cooling of methyl fluoride (CH3F). Molecules initially in 14 different rotational M-sublevel were pumped into a single M-sublevel, resulting in a molecule ensemble with over 70% of molecules in a single rotational state. More recently, we have achieved even better results for formaldehyde (H2CO), resulting in an ensemble with over 80% of molecules in the same rotational state.
R. Glöckner et al., Physical Review Letters 115, 233001 (2015)
A. Prehn et al., Physical Review Letters 116, 063005 (2016)
Interfacing polar molecules with Rydberg atoms
As a relatively new project, we have recently started investigating the combination of polar molecules with Rydberg atoms. Such a combination is interesting because both polar molecules and Rydberg atoms possess large electric dipole moments, allowing them to directly interact over micrometer scale distances. This promises to be useful for further improving control over polar molecules by for example allowing further cooling of the motional and internal molecular degrees of freedom. Moreover, a hybrid molecule-Rydberg-atom system would be a versatile toolbox for quantum simulations and quantum information processing.
Nondestructive detection of polar molecules via Rydberg atoms

A part of our interest in adding Rydberg atoms to our experiments originates in the possibility to solve a longstanding problem in our experiments: improving the detection of our molecules. In the past we have used commercial quadrupole mass spectrometers to detect our molecules, which is great because it is easy to apply to a wide range of different molecule species, but also results in low detection efficiencies on the order of 10-4. The strong interactions between polar molecules and Rydberg atoms should allow a much more efficient detection. Here, we have specifically proposed to detect molecules based on Förster resonant energy transfer. In Förster resonant energy transfer, a molecular excitation is transferred to the Rydberg atom (or vice versa) via the dipole-dipole interaction. This results in a state change of the Rydberg atom, which can easily be detected. A particular nice feature of such a detection is that it would be nondestructive, meaning that the molecules can be used for further experiments after they have been detected. First experiments are currently underway.
M. Zeppenfeld, Europhysics Letters 118, 13002 (2017)