QIP - Research Details
Quantum gates between atoms and photons
A single-sided high-finesse cavity can provide an interaction mechanism between single atoms and single photons. When an atom is strongly coupled to the cavity and a photon is reflected off this cavity, a π phase shift arises compared to the case with no atom coupled to the cavity. We exploit this mechanism to implement quantum gates between atoms and photons.
Atom-photon gate
When the coupling of the atom to the cavity is spin- and polarization dependent, the phase shift mentioned above can be exploited to realize a quantum logic gate between two qubits: the polarization state of the photon and the spin state of the single atom. The π phase shift conditioned on the state of the qubits results in a quantum gate that, in combination with single-qubit rotations, forms a universal set of quantum gates for quantum logic operations. One feature that distinguishes a quantum logic gate from a classical logic gate is the ability to create entanglement. We confirmed that the atom and the photon became entangled if both qubits are in appropriate initial states. Extending this scheme, we could even create three-party entanglement between the atom and two successively reflected photons.
A. Reiserer et al., Nature 508, 237 (2014)
Photon-photon gate
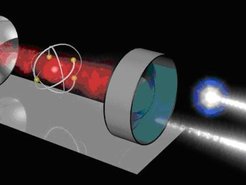
Photons hardly interact in vacuum, so that a photon-photon quantum gate requires matter to mediate the interaction. We thus used a single atom as an ancillary qubit to construct a photon-photon gate. After successive reflection of two photons from a cavity containing the single atom, we measured the atomic state in a suitable basis and applied classical feedback onto the state of the first photon to complete a deterministic gate protocol. Our gate will enable new applications in quantum in quantum information processing where photons are essential, particularly in quantum communication. The gate could also serve as the central processing unit of a scalable quantum computer.
B. Hacker et al., Nature 536, 193 (2016)
Application: Nondestructive detection of photons
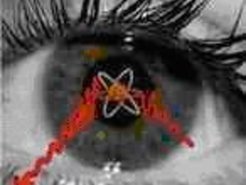
An application for the interaction mechanism described above is the nondestructive detection of a single photon. As usual, detection of a photon requires a detector whose state changes to an orthogonal state upon interaction with the photon. Conventional photodetectors accomplish this by absorbing the photon and making a transition to an excited state. Our nondestructive detector, however, employs a more subtle and genuine quantum mechanical effect. Instead of changing the energy of the detector by absorption, the photon flips the phase of a superposition state in which the detector is prepared. This phase flip occurs without energy exchange so that the photon is still alive after the interaction with the detector. This phase flip can be detected with atomic-state readout in a suitable basis using cavity-enhanced fluorescence. Thus, we can detect single, optical photons without absorbing them.
A. Reiserer et al., Science 342, 1349 (2013)
Quantum networks
Entanglement between a single atom and a BEC
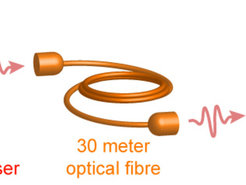
We experimentally generated remote entanglement between a single atom inside an optical cavity and a BEC. To this end, two laboratories joined forces. In one lab, a triggered single photon was created in an atom-cavity system, thereby generating entanglement between the internal state of the single atom and the polarization of the photon. The photon was transported in a 30 meter long optical fiber to the BEC in the other lab. Electromagnetically induced transparency was used to store this photon in the BEC in the form of a collective excitation. Here, the BEC served as a quantum memory for the photonic polarization qubit. The storage in the BEC established matter-matter entanglement. After a variable delay, the single photon was retrieved from the quantum memory. In addition, a second photon was generated from the single atom in the cavity, thereby mapping the internal state of the single atom onto the polarization of the single photon. These steps converted the entanglement onto the polarization states of two single photons. In the experiment, we found a total fidelity of all concatenated operations of 95 %. We observed a lifetime of the matter-matter entanglement of 100 µs, exceeding the photon duration by two orders of magnitude. The performance of the memory was independently measured using attenuated laser pulses, yielding an average process fidelity of unity with an error of only 0.004.
M. Lettner et al., Physical Review Letters 106, 210503 (2011)
An elementary quantum network
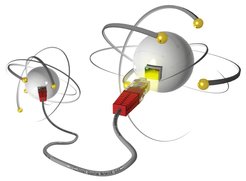
To demonstrate an elementary quantum network of identical nodes, we employed two independent atom-cavity systems spatially separated by 21 meters. Arbitrary qubit states are coherently transferred between the two network nodes in a conceptually very simple way: The quantum state of the sender node is mapped onto a single photon, which is then transmitted via an optical fiber and finally stored at the receiver node. By combining the generation of atom-photon entanglement with the storage of photonic qubits, we are able to create maximally entangled Bell states of the two atoms at distant nodes. The resulting nonlocal state can be manipulated by unitary operations applied locally at one of the nodes.
S. Ritter et al., Nature 484, 195 (2012)
Quantum teleportation
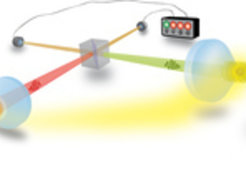
An alternative to the direct transfer of a quantum state via the exchange of a single photon is quantum teleportation. It has the advantage that it can be combined with quantum repeater schemes which allow for the creation of entanglement over arbitrary distances. We teleport quantum states of one atom onto another, again 21 meters apart. The protocol consists of three steps. First, entanglement between the receiver atom and a single photon is created. Second, the quantum state of the sender atom is mapped onto a second single photon and the Bell state of the two photons is measured. Third, a particular measurement result heralds a successful teleportation. The success probability of our cavity-based approach is almost five orders of magnitude larger than in previous experiments with remote material qubits. It is mainly limited by photon propagation and detection losses and could in the future be enhanced with a cavity-based deterministic Bell-state measurement.
C. Nölleke et al., Physical Review Letters 110, 140403 (2013)
Quantum memories
Single-atom quantum memory
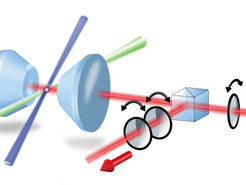
Quantum memories are the fundamental building blocks of quantum networks. We demonstrated the ability of single atoms to serve as such a memory by storing photonic quantum bits in a single atom. The quantum bit is encoded in the polarization of the photons. The polarization state is mapped onto the internal state of the atom by a stimulated Raman adiabatic passage. After a variable storage time, the photon is retrieved using a time-reversed version of the storage process. We characterized the fidelity, efficiency and coherence time of our quantum memory, demonstrating a storage fidelity of an unknown quantum state that outperforms any classical device.
H.P. Specht et al., Nature 473, 190 (2011)
Heralded storage of a photonic qubit in a single atom
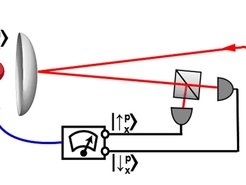
As the mapping processes between photons and quantum memories typically have efficiencies less than unity, and because photon propagation is associated with unavoidable losses, proper operation of a quantum network requires heralding events that signal successful completion of a protocol. Towards this goal, we implemented the heralded storage of a photonic polarization qubit in a single atom. The three-step protocol combines reflection of the photon from the atom-cavity setup, polarization-sensitive detection of the photon and feedback onto the atomic state. Detection of the photon heralds its presence and the successful state transfer. We also demonstrated the reverse process, namely transfer of the atomic state onto an impinging photon. The heralded storage mechanism is insensitive to fluctuations of most system parameters and features a record-high success probability of 39%.
N. Kalb et al., Physical Review Letters 114, 220501 (2015)
Next-generation cavity QED systems
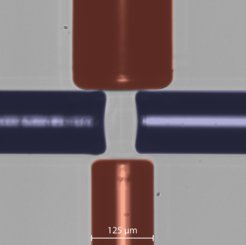
For the next generation of cavity QED experiments suitable for quantum information processing, we are developing fiber-based Fabry–Perot cavities with mirrors on laser-machined fiber end facets. These have two main advantages compared to the superpolished mirrors that were used in past setups: First, they feature smaller radii of curvature, resulting in a reduced mode volume for a given resonator length and thus a larger coupling strength to single atoms. Second, the small size of the optical fibers used as mirror substrates allows for geometric arrangements of several cavities in close proximity that would not be possible with larger mirrors. We use a CO2 laser to evaporate the glass in the center of a fiber end facet to machine near-spherical concave depressions with radii of curvature in the range of 80-600 µm. Due to surface tension, the resulting surface is very smooth and therefore suitable as a mirror substrate. After the application of a highly reflective coating, cavities with a finesse of up to 190000 were built from two of these mirrors.
The crossed-cavity setup
We have recently used this new generation of fiber-based cavities in order to set up a new experiment with single Rubidium atoms trapped at the center of two crossed fiber-based Fabry-Perot cavities. The crossed cavity setup is placed in a chamber with ultra-high vacuum conditions and combined with other components that allow us to control, manipulate and measure the properties of the single atom. Some of these components include laser beams and electromagnetic coils that are used to cool and trap the atom, an imaging system that collects the light emitted by the atom into a camera in order to determine it’s presence and position, or antennas that send microwave radiation in order to manipulate the atom’s internal state. We can trap atoms for several tens of seconds at the crossing point of the two cavity modes achieving a regime of high atom-photon cooperativity with both cavities. One of the main objectives of this platform is the development of novel quantum information processing schemes based on two-mode cavity QED.
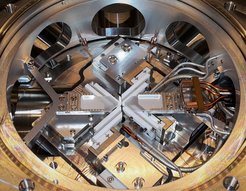

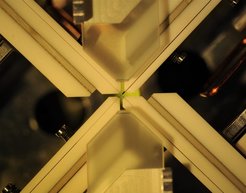
A passive heralded quantum memory
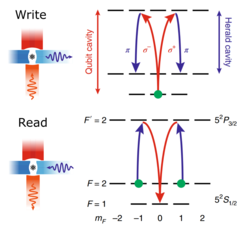
Exploiting the new possibilities given by the two cavities, we have recently investigated a new scheme enabled by our two-mode cavity QED setup. We implemented a quantum memory able to store photonic polarization qubits with two very interesting properties: (1) the memory is passive, meaning that no laser field needs to be applied during the storage process, and (2) the successful storage is heralded by the emission of a single photon, which enables the mitigation of storage errors and to improve qubit fidelities. This new quantum memory scheme was realized by placing one cavity in an atomic transition suitable for the interaction of the single atom with the photonic qubit, and the other cavity in a transition suitable for photon-to-atom qubit transfer while generating the herald photon. The qubit could then be stored for some time in the atom and be subsequently mapped back into another photon using the interaction of the atom with the two cavity modes. The passive photon-to-atom qubit transfer was enabled by an engineered polarization-mode frequency splitting in one of the two cavities. This photonic quantum memory will be important in order to realize quantum information network tasks where the heralding and storage of photonic qubits is important, such as for quantum repeaters
M. Brekenfeld et al., Nature Physics 16, 647-651 (2020)
Nondestructive detection of photonic qubits
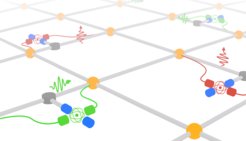
Qubits encoded in single photons are very important to distribute quantum information over remote locations, but at the same time are also very fragile objects. The loss of photonic qubits (through absorption, diffraction or scattering) is actually the main limitation in the maximum reachable quantum communication distance. In this context, the nondestructive detection of photonic qubits is a great scientific challenge that can help tracking the qubit transmission and mitigate the loss problem. We have used our crossed-cavities coupled to a single atom as a very subtle detector that employs quantum mechanics effects to detect single photon qubits without destroying the photon nor the encoded quantum information. The photonic qubits are reflected in one of the resonators while leaving a trace on a quantum superposition state of the atom. This change in the atomic superposition state occurs without energy exchange, such that the photon qubit is still alive after the interaction with the detector. The change in the atomic state can be then measured in a suitable basis using cavity-enhance fluorescence with the second crossed resonator, indicating the presence of the reflected photonic qubit. We characterized the performance of the nondestructive detector and have shown that with the current parameters it can already provide an advantage and in different quantum communication scenarios.
D. Niemietz et al., Nature 591, 570–574 (2021)
Controlling the frequency splitting of polarization eigenmodes
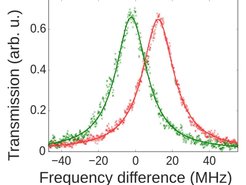
It has been observed by several research groups that microscopic Fabry–Perot cavities are prone to an increased frequency splitting of polarization eigenmodes compared to macroscopic mirrors. We have shown that this effect is caused by a non-zero eccentricity of the mirrors. It is explained by corrections to the paraxial approximation, which become relevant for microscopic mirrors and result in a phase shift between two linear polarization eigenmodes. We derived a simple approximate expression for this effect, which matches our experimental observations and can be used to calculate the expected phase shift of a mirror from its geometry. We also demonstrated that the frequency splitting can be controlled by rotating one of the mirrors around the cavity axis. When two mirrors with opposite phase shift are used, the round-trip phase shift can be close to zero, resulting in near-degenerate polarization eigenmodes.
M. Uphoff et al., New Journal of Physics 17, 013053 (2015)
Towards a quantum repeater
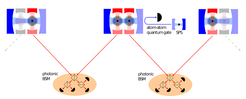
We have also theoretically investigated the potential of fiber-based Fabry–Perot resonators coupled to single atoms. A particularly interesting application is a quantum repeater node. Such a device that would enable the distribution of entanglement over large distances requires long-lived quantum memories and efficient coupling of these memories to photonic qubits. Single atoms in optical cavities are prime candidates to fulfill these criteria and could be used to implement a quantum repeater protocol. We have proposed an implementation of a quantum repeater at telecom wavelength that focuses on a realistic scenario based on highly integrated and highly efficient fiber cavities. Numerical simulations confirm that such a quantum repeater could outperform direct entanglement over distances exceeding 50 km, under the assumption of realistic parameters derived from the results of our fabrication of fiber-based Fabry–Perot resonators.
M. Uphoff et al., Applied Physics B 122, 46 (2016)
Beyond single atom cavity QED
Many quantum information processing protocols require several atoms, instead of just a single one, inside an optical resonator. Motivated by such proposals, we started to explore experimentally the radiative properties of a pair of atoms coupled to the same cavity mode.
Interference of light emitted by an atom pair
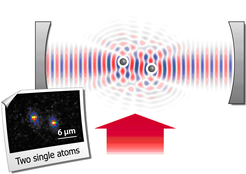
An optical lattice provides good localization of the atoms. In combination with a high-numerical-aperture microscope objective, we determined the distance between two atoms with sub-wavelength resolution. We then studied how light from an excitation laser is scattered into the cavity and observed nontrivial interference phenomena that depend on the phase difference with which the atoms couple to the driving laser and the resonator mode. The scattering rate depends on this phase in a sinusoidal way, much like in the absence of a resonator. However, the presence of the resonator distinctly reduces the contrast of the interference pattern. Moreover, the photon pair-correlation function of the scattered light exhibits striking features like a giant photon-bunching effect that occurs when the two atoms are at a distance where they emit into the cavity with opposite phase. This behavior is explained in the framework of the Tavis-Cummings model.
A. Neuzner et al., Nature Photonics 10, 303 (2016)
Quantum control of single atoms inside a cavity
Controlling quantum dynamical processes is the key to practical applications of quantum physics, for example in quantum information science. The manipulation of light-matter interactions at the single-atom and single-photon level can be achieved in Cavity-QED, in particular in the regime of strong coupling in which the atom and cavity form a single entity. In the optical domain, this requires a single atom at rest inside a microcavity.
Trapping and cooling of a single atom inside a cavity
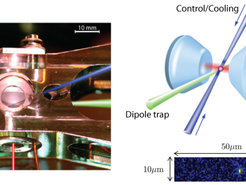
We have developed an experimental setup consisting of an orthogonal arrangement of cooling lasers, trapping lasers and a cavity, which gives rise to a unique combination of friction forces acting along all three directions. This technique has been applied to optically cool single atoms in a high-finesse cavity with high efficiency which leads to an average single-atom trapping time of several tens of seconds, during which the strongly coupled atom can be observed continuously. The experimental sequence starts by preparing a cloud of cold Rubidium atoms in a magneto-optical trap (MOT). They are subsequently loaded into a horizontal dipole trap beam and guided into the cavity. The position of the atom in the cavity can be precisely controlled and adjusted using a standing-wave dipole trap. Then we use the trap as a conveyor belt that we set into motion perpendicular to the cavity. This allows us to repetitively move atoms out and back into the cavity mode with a repositioning precision of 135 nm. This makes it possible to either selectively address one atom of a string of atoms by the cavity, or to simultaneously couple two precisely separated atoms to a higher mode of the cavity. Absorption images of the trapped atoms with a resolution of 1.2 µm allow for the reliable determination of the number of trapped atoms.
S. Nußmann et al., Nature Physics 1, 122 (2005)
S. Nußmann et al., Physical Review Letters 95, 173602 (2005)
Ground-state cooling
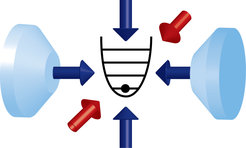
We extended the trapping geometry to a three-dimensional optical lattice formed by one red- and two blue-detuned standing waves and thereby dramatically increased the confinement of the atoms. This puts our system into the strong-coupling regime of cavity QED. By Raman sideband cooling of the atom, we achieve an occupation of the three-dimensional ground state of 89%. The good position control opens up the possibility to use the atomic motion as a quantum register.
A. Reiserer et al., Physical Review Letters 110, 223003 (2013)
Resonance shifts in deep optical lattices
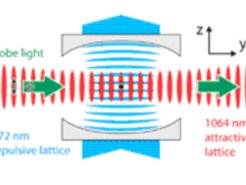
The quasi-permanent trapping of single atoms in optical cavities is crucial for many applications in quantum information processing. Optical lattices formed by far-detuned standing-wave dipole traps are particularly successful as they combine good position control and tight confinement of the atom with easy integration into the cavity setup. As a typically undesired by-product, dipole traps shift the atomic resonance frequencies. Knowledge of the exact light shifts caused by these traps is therefore essential for driving transitions resonantly. In contrast to the linear dependence of the light shift on the light intensity in shallow traps, we observed a nonlinear dependence in a deep trap. The reason for this is the breakdown of hyperfine coupling between nuclear spin and angular momentum of the valence electron. This also explains our experimental observation that inside a deep trap we can easily drive dipole-forbidden transitions. This has severe consequences for experimental schemes relying on the forbidden character of certain transitions. Our data also yield values for the scalar and tensor polarizabilities of 87Rb at 1064 nm of one of the excited states.
A. Neuzner et al., Physical Review A 92, 053842 (2015)
Lossless State Detection of Single Neutral Atoms
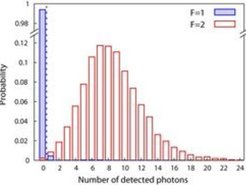
Detection of the quantum state of a single atom is at the basis of atomic physics. Moreover, such a readout of a single atom’s internal state is highly relevant for quantum information science, as this is required for the implementation of quantum processors. This quantum information may be encoded, for example, in atomic hyperfine states. However, the readout of the hyperfine quantum bit has proven remarkable difficult for neutral atoms and is usually destructive – much like a hard drive which is destroyed after reading from it just once! In this work, we solve this problem and show that by coupling a single rubidium atom to a high-finesse cavity we can establish a superior readout channel for internal atomic states. Our scheme is based on cavity-enhanced fluorescence detection and makes use of the Purcell effect. It has proven very robust against experimental parameter variations. We achieve a hyperfine state detection fidelity of 99.4 % within a readout time of only 85 µs. Most important, the atom can be interrogated many hundred times without loss from its optical dipole trap. This presents an essential advancement for the speed and scalability of quantum information protocols based on neutral atoms and establishes single neutral atoms as truly stationary carriers of quantum information.
J. Bochmann et al., Physical Review Letters 104, 203601 (2010)
Earlier experiments on light-matter quantum interfaces
Controlled generation of single photons with one atom
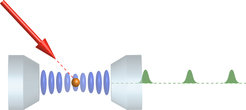
Deterministic single-photon sources are of prime importance in quantum information science. Such sources have been realized with neutral atoms, embedded molecules, trapped ions, quantum dots and defect centers. All of these sources are suitable for applications where the indivisibility of the emitted light pulses is essential. For quantum computing or quantum networking, the emitted photons must also be indistinguishable, which has been demonstrated with single trapped atoms. Another requirement is a high efficiency. This is hard to obtain in free space, as typical light-collecting lenses cover only a fraction of the full 4π solid angle. The efficiency can be boosted by strongly coupling the radiating object to an optical microcavity, as has been achieved with atoms, quantum dots and ions. An additional advantage of the cavity is that a vacuum-stimulated Raman adiabatic passage (vSTIRAP) can be driven in a multilevel atom. In this way, the amplitude, frequency and polarization of the photon can be controlled.
We have implemented a cavity-based scheme with a dipole laser for trapping, a trigger laser for photon generation and a recycling laser for repumping, monitoring and cooling the atom. The scheme combines high photon-generation efficiency of up to 60% and long trapping times. Two remarkable features are, first, that the single-photon stream is specified by its intensity correlation function evaluated in real-time during a short time interval after system preparation and, second, that its subsequent performance is guaranteed by monitoring the atom without perturbing the single-photon stream.<
M. Mücke et al., Physical Review A 87, 063805 (2013)
T. Wilk et al., Physical Review Letters 98, 063601 (2007)
M. Hijlkema et al., Nature Physics 3, 253 (2007)
A. Kuhn et al., Physical Review Letters 89, 067901 (2002)
Phase control of single photons
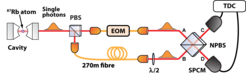
While the phase of a coherent light field can be precisely known, the phase of the individual photons that create this field, considered individually, cannot. Phase changes within single-photon wave packets, however, can be observed. Our single photon sources allow to tailor the photonic bandwidth. Theresulting long photonic wave packets enable the high speed phase modulation and detection of the photon shape, opening interesting avenues for research. In our lab, we have developed this technology to modulate the phase of a single photon wave packet. The phase modulation is applied via a fiber electro-optical modulator (EOM) during the passage of individual photons without affecting the photon’s amplitude profile. The resulting phase is verified via a two-photon fourth-order quantum interference measurement. If no modulation is introduced, typical Hong-Ou-Mandel behavior is expected. However, if a phase change is applied within the photon envelope, the coalescence behavior of the interference is altered, which can result in the fermionic spatial behavior of photon pairs. Combined with previously demonstrated control of a single photon’s amplitude, frequency, and polarization, the phase shaping presented here allows for the complete control of single-photon wave packets.
H. P. Specht et al., Nature Photonics 3, 469 (2009)
Fast excitations and single photons
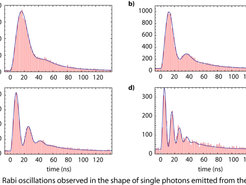
In addition to vacuum-stimulated Raman adiabatic passage, fast excitation of a single intracavity atom can create single-photon wave packets of well defined frequency, polarization and shape. Furthermore, if the excitation pulse is fast compared to all other processes (atom-cavity coupling strength, atomic polarization decay rate, and cavity field decay rate) it will result in a near-instantaneous promotion of the atom to the excited state. The coupled atom-cavity system evolves coherently with an oscillatory energy exchange between the atom and the cavity - a phenomenon known as vacuum Rabi oscillation. An intriguing feature of this excitation scheme is that the wave packet of a single emitted photon is governed by the normal-mode spectrum of the coupled atom-cavity system, independent of the excitation pulse shape and frequency. This excitation scheme allows for the rapid generation of single photons on cycling transitions, not possible with the vSTIRAP technique.
J. Bochmann et al., Physical Review Letters 101, 223601 (2008)
Quantum beat of two single photons
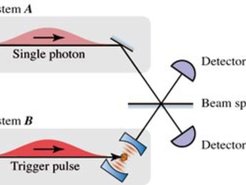
The quantum nature of light impressively manifests itself in the fourth-order interference of two identical and mutually coherent single photons that impinge simultaneously on a beam splitter. Two initially completely independent photons coalesce and both leave the beam splitter in the same direction. To investigate the temporal dynamics behind this interference phenomenon, we use the adiabatically driven, strongly coupled atom-cavity system described above as a single-photon emitter. Photons are generated by a unitary process, so that their temporal and spectral properties can be controlled. In fact, the duration of the photons used in our experiment exceeds the time resolution of the employed single-photon counters by three orders of magnitude. As a result, we observe interference of the two photons even if their relative frequency difference exceeds their bandwidths.
T. Wilk et al., Physical Review Letters 98, 063601 (2007)
T. Legero et al., Physical Review Letters 93, 070503 (2004)
Electromagnetically induced transparency with single atoms in a cavity

Electromagnetically Induced Transparency (EIT) is an effect that allows the optical properties of an atomic medium to be radically changed by means of light. Previously, scientists have studied this effect, using atomic ensembles with hundreds of thousands of atoms. In our system, we have used this effect to control the optical response of a single atom. The atom is trapped inside the high-finesse optical cavity and the transmission of a probe laser incident on the cavity axis is measured. The presence of the atom causes the light to be reflected, and the transmission drops. With an additional control laser applied transverse to the cavity axis, the single-atom EIT condition is achieved and maximum transmission is recovered. The single atom effectively acts as a quantum optical transistor, coherently controlling the transmission of light through the cavity. These results provide a considerable milestone in the control of matter and light at the fundamental level, since it marries for the first time single-atom, strong-coupling cavity quantum electrodynamics and EIT. This could bring low-loss, giant optical nonlinearities into the realm of both single photons and single atoms, opening enormous possibilities towards the development of photonic quantum gates.
M. Mücke et al., Nature 465, 755 (2010)
Atom-Photon Entanglement
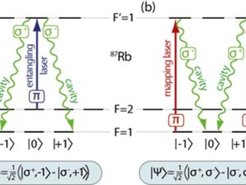
Entanglement is a fundamental resource in quantum information science. In particular, entanglement of a long-lived massive particle and a flying photon for the purpose of quantum communications and quantum networking must be performed with high efficiency. This makes the realization of a quantum interface between such particles and the efficient generation of entangled photons for quantum repeaters an important goal. Our setup provides a deterministic scheme to create entanglement between a single trapped atom and a single photon. In a second step the atomic superposition state can be mapped onto another photon. The latter step disentangles the atom from the light producing two entangled photons.
Our protocol produces a sequence of single flying photons emitted from a single 87Rb atom in a high-finesse optical cavity. The polarization state of the first emitted photon is entangled with the internal state of the atom. In contrast to previous experiments with single ions or atoms, the atomic state is not examined via a shelving technique and detecting fluorescence photons. Instead, our scheme maps the atomic state onto the state of a second single photon. As a result of the state mapping a pair of entangled photons is produced, one emitted after the other into the same spatial mode. The state of the photons is analyzed by tomography of their polarization states, which also probes the prior entanglement between the atom and the first photon.
B. Weber et al., Physical Review Letters 102, 030501 (2009)
T. Wilk et al., Science 317, 488 (2007)
Financial Support and Open Positions
This project is financially supported by the BMBF via the Q.com project. Previous funding came from DFG Research Unit 635, the European Union via Integrated Projects SIQS, AQUTE and HANAS, the state of Bavaria within the Doktorandenkolleg ENB-QCCC, and the BMBF via the QuOReP project.
If you want to join our team, please visit our Open positions page.