Our experimental approach: Using Erbium-doped crystals in optical resonators
To realize large quantum networks, we will employ the spin of impurities in a solid that can give access to long coherence times. This can be achieved either by using host crystals that provide a “magnetic vacuum” [1], i.e. that are free of other paramagnetic impurities and made of nuclei without a spin. Alternatively, one can operate under specific experimental conditions in which the impurity spin transition frequency is not sensitive to perturbing magnetic fields. The latter approach has enabled the current world record of six hours in quantum coherence time using Eu3+ ions in Yttrium Orthosilicate (Y2SiO5) [2].
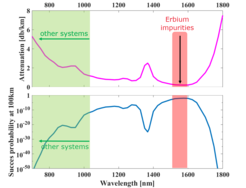
In our group, we use a different emitter: Erbium (Er3+) dopants. The main reason for this choice is that it is the only known emitter that exhibits coherent optical transitions within the "telecommunications" wavelength regime around 1500 nm. This not only allows us to reduce the experimental overhead by harnessing existing photonic technologies. More important, the loss of photons in optical fibers is minimal at these wavelengths (see figure): compared to the visible, the transmission over 100 km of optical fiber is increased by about 60 orders of magnitude! This property of our system is a unique advantage and seems mandatory for the implementation of quantum networks that span global distances.
Similar to other rare-earth impurities, Erbium dopants can exhibit cohrerence times in the range of seconds [3], sufficient to send photons many times around the earth. Such exceptional coherence times can be achieved by encoding quantum bits using the spin of electrons in inner-shell 4f levels, which are largely shielded from the electric fields of the crystal by the outer shell 5s and 5p electrons [4]. The resulting small coupling to phonons can lead to long ground state spin lifetimes even at elevated temperatures. Therefore, rare-earth doped crystals were investigated already back in the seventies towards classical data storage. In spite of these early experiments, their use towards quantum rather than classical information processing has only emerged recently, mainly in experiments that employ large ensembles of ions [2, 3]. Unfortunately, the absence of techniques to perform local deterministic quantum gate operations hinders the realization of a quantum repeater with such ensembles.
In contrast, such operations are well-developed for single dopants. Still, their use for quantum networks has not been shown so far because the long-lived optical transitions (with lifetimes of several ms) lead to a very small fluorescence rate, which makes the optical detection, coupling and readout of individual ions challenging. Our group overcomes this challenge by embedding Erbium-doped crystals into optical resonators [5] that are formed either by conventional mirrors or by nanophotonic structures. In such setting, the excited state lifetime of Erbium ions can be strongly reduced by the Purcell effect, and the emission of individual ions is efficiently channeled into an optical output mode. This facilitates both efficient qubit readout and coupling between remote ions, which is a critical enabler for the development of global quantum networks.
[1] Saeedi, K. et al. Room-Temperature Quantum Bit Storage Exceeding 39 Minutes Using Ionized Donors in Silicon-28. Science 342, 830–833 (2013).
[2] Zhong, M. et al. Optically addressable nuclear spins in a solid with a six-hour coherence time. Nature 517, 177–180 (2015).
[3] Rančić, Hedges, Ahlefeldt, and Sellars: Coherence time of over a second in a telecom-compatible quantum memory storage material. Nature Physics 14, 50 (2018).
[4] Thiel, Böttger, Cone, Rare-earth-doped materials for applications in quantum information storage and signal processing. Journal of Luminescence 131, 353–361 (2011).
[5] Reiserer and Rempe: Cavity-based quantum networks with single atoms and optical photons. Rev. Mod. Phys. 87, 1379 (2015); Merkel, Ulanowski, and Reiserer: Coherent and Purcell-Enhanced Emission from Erbium Dopants in a Cryogenic High-Q Resonator, Phys. Rev. X 10, 041025 (2020).